Research activities
Phase diagram of QCD matter
Phase diagrams aim in describing the physical states of a given substance under different conditions e.g. of pressure and temperature. We are very much familiar for example with the phase diagram of the water, a simplified version of which is depicted in the left plot of the figure below in the pressure (P) and temperature (T) plane. This diagram has been studied in quite some detail and allowed us to distinguish the various phases of water (i.e. gas, liquid, solid), the solid lines that represent the phase boundaries between the three phases, but also the points where phase transitions take place.
In a similar way, the phases of QCD matter can be depicted in the QCD phase diagram, where usually temperature and baryo-chemical potential are now in the two axis. An example of such a diagram is given in the right plot of the figure above. In contrast to the case of the water, the phase diagram of QCD matter is not known to great detail but mainly at a qualitative level, with the only exception being the area at vanishing values of μB and large T. The various regions of QCD matter, some discussed below, are separated by boundaries whose position is not known experimentally in a precise way. Actually, the QCD phase diagram may be richer than what is shown here.
At low values of temperature and at ranges of μB between few hundreds of MeV to few GeV one encounters normal hadronic and nuclear matter. Once this matter is heated and compressed the main building blocks of ordinary matter, the quarks and gluons, become deconfined in a state of matter called quark gluon plasma (QGP) i.e. the “hot” and dense QCD matter.
Apart from the region of hadrons, the “cold” QCD matter at finite μB and the hot and dense region of quarks and gluons, there is also a region characterized by cold QCD matter at extremely dense conditions i.e. at high values of μB characterizing matter in the interior of stellar objects such as neutron stars.
Hot and dense QCD matter: Studying the quark gluon plasma with heavy-ion collisions
ALICE is the dedicated heavy-ion detector at the LHC. It consists of a number of detector subsystems that provide vertex determination , tracking and particle identification over a wide range of momentum.
My research interests are focused on measurements of:
- particle correlations such as flow studies
- fluctuations of conserved quantities
- heavy flavor production
I am also currently involved in the upgrade of the Inner Tracking System (ITS) of ALICE, scheduled to be installed during the LS2 as well as to preliminary studies about the new heavy ion detector planned to be operated after 2030 at the LHC.
Over the last decades scientists try to reproduce the QGP and study its properties by colliding heavy ions at very high energies. There are several experimental facilities that were used, such as the Super Proton Synchrotron (SPS) at CERN, the Relativistic Heavy-Ion Collider (RHIC) at BNL, and nowadays the Large Hadron Collider (LHC) at CERN. The latter, apart from delivering collisions of protons on protons, collides lead on lead ions at very high energies allowing all four major experiments, ALICE, ATLAS, CMS and LHCb to study the properties of this primordial state of matter, the QGP.
Cold and dense QCD matter: Equation of State (EoS) of neutron stars via gravitational waves
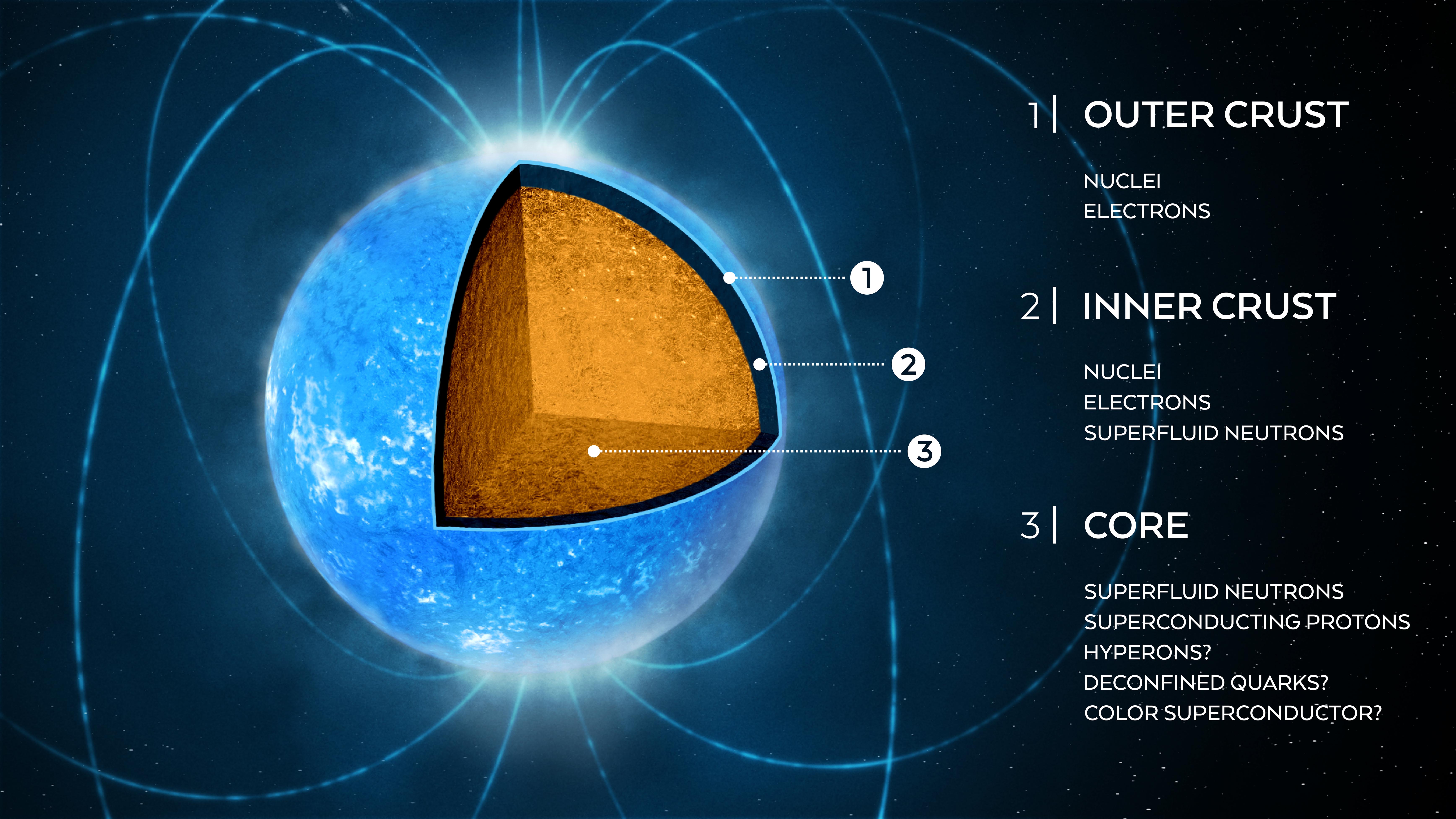
Gravitational waves are ripples in space and time caused by massive objects, e.g. neutron stars, black holes, moving with extreme accelerations. These objects orbit around one another at increasing rates.
Albert Einstein predicted the existence of gravitational waves in 1916 in his general theory of relativity. Einstein's mathematics showed that massive accelerating objects (such as neutron stars or black holes orbiting each other) would disrupt space-time in such a way that 'waves' of distorted space would radiate from the source (like the movement of waves away from a stone thrown into a pond). Furthermore, these ripples would travel at the speed of light through the Universe, carrying with them information about their cataclysmic origins, as well as invaluable clues to the nature of gravity itself.
It was recently realized that gravitational waves give access to the Equation of State (EoS) of some of the most remarkable stellar objects in the universe i.e. neutron stars. This can be done by studying the inspiral of binary neutron stars due to gravitational radiation. The tidal distortion of neutron stars in a binary system links the EoS of the cold and dense QCD matter to the gravitational wave emission during the inspiral. On the 17th of August 2017, this expectation was put into test with the observation of gravitational waves by both the Ligo and Virgo collaborations coming from the coalescence of a neutron star binary system.